In the 2015 Pixar film Inside Out, a young girl’s mind is brought vividly and hilariously to life through a cast of animated archetypes, each representing a distinct facet of emotion or thought. The brain, in this portrayal, is a modular structure, composed of interlocking yet discrete parts — a concept that mirrors one of the most advanced models in modern neuroscience.
Over the past decade, researchers have coaxed stem cells to form 3D clusters of neural tissue known as organoids, bathing the cells in carefully formulated chemical cocktails to generate brain regions such as the thalamus, where sensory information is processed, and the striatum, which integrates movement and reward signals.
Initially, efforts focused on isolated, region-specific models. But today, researchers routinely fuse organoids representing distinct brain areas to create unified ‘assembloids’, capable of reconstructing functional neural networks and offering insights into brain connectivity, development and disease. “It has opened a new direction in the field to really understand interactions between different parts of the brain,” says In-Hyun Park, a stem-cell biologist at the Yale School of Medicine in New Haven, Connecticut.
These 3D model brains with cells from several people are first of their kind
Yet the brain is more than a patchwork of interconnected structures, stuck together like Lego bricks. It is a dynamic, self-organizing ecosystem in which neighbouring cells continuously shape one another’s fate. Over time, an intricate web of synaptic pathways emerges through fine-tuned exchanges — both local and long-range — that help all the parts of the organ to mature in synchrony.
No one organoid model can capture this complexity. Assembloids provide a valuable window into regional interactions. But techniques are emerging to recapitulate further aspects of brain mechanics and circuit dynamics. From neurodevelopment to drug discovery, organoid models are opening up fresh investigative paths, each geared to different dimensions of brain biology — and with tools suited to a variety of lines of scientific inquiry.
“We cannot really talk about ‘best’ models, but rather models that are more adequate to answer specific questions,” says Silvia Velasco, a developmental neurobiologist at the Murdoch Children’s Research Institute in Parkville, Australia. And because our understanding of human brain development is “still largely fragmented”, Velasco adds, a diverse modelling toolkit is not just helpful — it’s essential.
Diversity, however, comes with trade-offs. Greater biological realism often means lower scalability and reproducibility, especially between research groups. This complicates efforts to draw broad conclusions and translate discoveries reliably from the laboratory to the clinic. Still, researchers such as Paola Arlotta, a neuroscientist at Harvard University in Cambridge, Massachusetts, are working to close the gap. “We need models that have fidelity,” she says.
Network effects
In Arlotta’s lab, tiny white globs of brain cells swirl in a cerise-coloured bath of growth medium, like ‘pearls’ in a cup of hibiscus bubble tea. These are three-month-old organoids, made from human stem-cell-derived neural precursors that, together, form the cerebral cortex, the wrinkled outer layer of the brain. Each organoid comes from a population of carefully patterned stem cells, combined in just the right amounts and cultured over several months to create microcircuits. The result is a balanced mix of excitatory and inhibitory neurons that have developed in unison, forming intricate, functional networks.
“They are really growing together,” exclaims Irene Faravelli, a neurologist in Arlotta’s group. After years of tinkering to get the conditions just right, “now we are at the fun part of exploring what we can interrogate with this model”, she says. “We can ask about the mechanisms controlling the formation of the human cerebral cortex, the site of highest cognitive function. Or dig deeper into how the unique features of the human brain are acquired and what makes our brain different.”
Mini-colon and brain ‘organoids’ shed light on cancer and other diseases
Most importantly, she adds, the model lets researchers probe the way in which genes linked to conditions such as autism, schizophrenia and Alzheimer’s disease shape brain development and function.
Last year, Arlotta, Faravelli and their colleagues described a reliable method for growing these composite organoids — dubbed chimeroids. But at the time, instead of mixing cells from different brain regions, the researchers pooled a single kind of neural precursor cell from donors1.
Now, in unpublished follow-up work, they have adapted these cultures to start from a mix of progenitors corresponding to different brain regions. These are the spheres that Faravelli now gently coaxes along in the lab.
The goal, explains Arlotta, is to recapitulate cell–cell interactions that occur as the brain naturally develops — mimicking the choreography of cellular differentiation, migration and connectivity that unfolds as cells mature in a shared environment. “This is how the nervous system develops,” she says.
“I like to think about the process of building a brain much like an orchestra producing a specific musical symphony,” Arlotta continues. “Much like instruments playing at the wrong times and producing a different music, cells that do not interact with each other correctly during development will produce a different final tissue.”
Assembloid models created by physically joining pre-formed brain organoids can also recapitulate some of the same circuit-level interactions — and Arlotta is quick to note that chimeroids and assembloids are complementary. But to investigate the earliest stages of brain development, she realized, a different approach was needed: hence, chimeroids.
“It’s the only way you can watch these intricate processes of cell–cell interaction and communication that progressively build our beautiful brain,” Arlotta says.
Model assembly
The careful calibration of timing and cell composition needed to engineer chimeroids stands in contrast to one of the field’s most high-profile early successes.
In 2013, a team led by developmental neurobiologists Madeline Lancaster and Jürgen Knoblich at the Austrian Academy of Sciences’ Institute of Molecular Biotechnology in Vienna showed that human stem cells, when embedded in a gelatinous protein matrix and cultured in spinning bioreactors, could spontaneously assemble into 3D, multilayered brain-like structures with discrete regions and architectural patterns2.
A triumph of self-organization, these structures, with features akin to those found in the brain of a nine-week-old human fetus, provided an unprecedented window into neural development and disease — including microcephaly, a condition marked by severely impaired brain growth that is difficult to replicate in animal models. “It’s not just one small part of the brain” in these organoids, says Lancaster, who is now at the MRC Laboratory of Molecular Biology in Cambridge, UK. “It’s actually this whole complex make-up of different brain regions connected to each other.”
Organoids open fresh paths to biomedical advances
Knoblich vividly recalls the reaction when he first presented the work at a scientific conference in 2012, ahead of publication. He was a last-minute replacement for another speaker and had no idea what to expect. Neither did the crowd — which erupted with excitement. “Never in my life have I given a talk that created this kind of attention,” Knoblich says. “It was amazing.”
Other researchers soon started adapting and refining the technique, showcasing its potential for studying complex neural circuits and disease mechanisms. But the self-organizing nature of these early models meant that variability was baked in. No two organoids were identical, which made reproducibility a persistent challenge. Researchers addressed this by introducing different growth factors and signalling molecules that control cell fate and tissue formation more tightly.
First came protocols for making just part of the cerebral cortex. Methods for generating midbrain and hypothalamic organoids soon followed, along with techniques for modelling the cerebellum, spinal cord and other structures — each requiring its own set of signalling cues and culture conditions, tailored to mimic region-specific development.
As the fidelity of region-specific brain organoids improved, researchers embraced a new frontier: modelling the connections between them. This led to the development of assembloids — hybrid constructs that recreate the functional wiring of the brain and offer a deeper look into its integrative architecture.
“It’s all about complex cell interactions that give rise to emergent properties,” says Sergiu Pașca, a neuroscientist at Stanford University in California who coined the term ‘assembloid’ and led one of three teams that independently introduced the concept in 20173–5 (Knoblich and Park led the other two).
Neural knotwork
Assembloids allowed scientists, for the first time, to observe long-range neuronal crosstalk in a dish — such as the migration of inhibitory neurons from the ventral forebrain into the dorsal cortex — thereby recapitulating key events in fetal brain development. “No one knew how that migration was actually happening in humans,” says Pașca. Assembloids also created a powerful platform for studying neuropsychiatric conditions such as Rett syndrome, epilepsy and schizophrenia, in which circuit-forming events go awry.
Knoblich credits the widespread adoption of assembloids to their mix-and-match nature. “There’s not much to it,” he says. “You take two organoids, you put them together, and they fuse — that’s it.”
“The challenge,” he points out, “is to get the right interaction.”
Human brain cells implanted in rats prompt excitement — and concern
In 2020, Paşca’s lab introduced three-part assembloids, fusing cortical, spinal-cord and muscle organoids to study how neural activity triggers muscle contractions6. Now, the team has pushed the approach even further. By stitching together organoids carrying neurons involved in each key stage of pain processing — from peripheral sensory neurons to the spinal cord, through to the thalamus and finally the cortex — the team was able to recapitulate the entire pathway and even watch it respond to a noxious stimulus such as capsaicin, the compound responsible for the spicy heat in chillies.
What’s more, the Stanford researchers demonstrated last month how heritable mutations in a key signal-relaying protein can profoundly alter these pain-transmission dynamics7. This helps to explain why such mutations can, depending on the nature of the genetic alteration, lead to either complete insensitivity to pain or a hypersensitivity disorder marked by sudden, intense burning or stabbing pain in the rectum, eyes and jaw.
Making the connection
A complementary approach to studying how brain regions interact comes from Yoshiho Ikeuchi, a bioengineer at the University of Tokyo. Instead of fusing organoids, Ikeuchi and his colleagues connect them using tiny plastic channels on a microfluidic device. This set-up keeps the organoids physically separated and encourages nerve fibres to close the gap, mimicking the long-distance communication patterns seen in the human brain8.
In unpublished work, Ikeuchi has connected as many as 18 organoids in a 6 × 3 grid — an effort he admits was driven more by curiosity than by a specific objective. “It’s so fun to watch how they generate spontaneous activity and respond to our stimulation,” he says. But, beyond the novelty, Ikeuchi sees real potential in using these ‘connectoids’ to study diseases such as schizophrenia, which involves disruptions in axonal function and synaptic integrity.
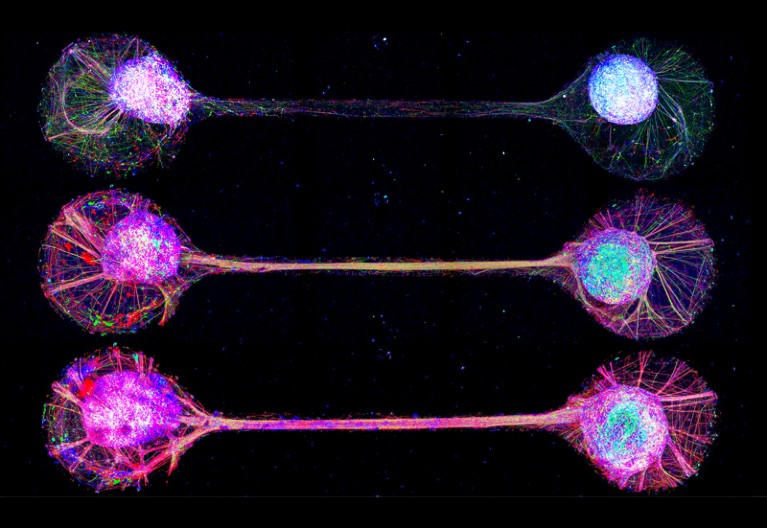
‘Connectoids’ use microfluid channels to recapitulate and study neural connections.Credit: Institute of Industrial Science, The University of Tokyo
Connectoids also provide a way to study communication across the corpus callosum, the physical barrier that separates the two hemispheres of the brain. Last year, in collaboration with Knoblich’s lab, Ikeuchi and his colleagues used paired organoids connected through microchannels to show how a gene mutation associated with intellectual disability disrupts the formation of long-range axonal projections, leading to connectivity defects9.
Although physical fusion and engineered connections can help to simulate neural communication, a true understanding of how these interactions emerge during early brain development requires turning back the biological clock. That means guiding not just the final position of cells, but also the paths they take to get there — a process orchestrated by signalling molecules known as morphogens.
Increasingly, brain-organoid researchers are turning to microfluidics to harness these molecules with precision. By exposing developing tissues to carefully calibrated gradients of morphogens, researchers such as Flora Vaccarino, a developmental neurobiologist at Yale, have managed to steer neural precursor cells towards specific identities in a spatially organized way. “You kind of trigger what the organoids will be, and then it’s a self-organizing system,” says Vaccarino. “Everything else is like a chain of events.”