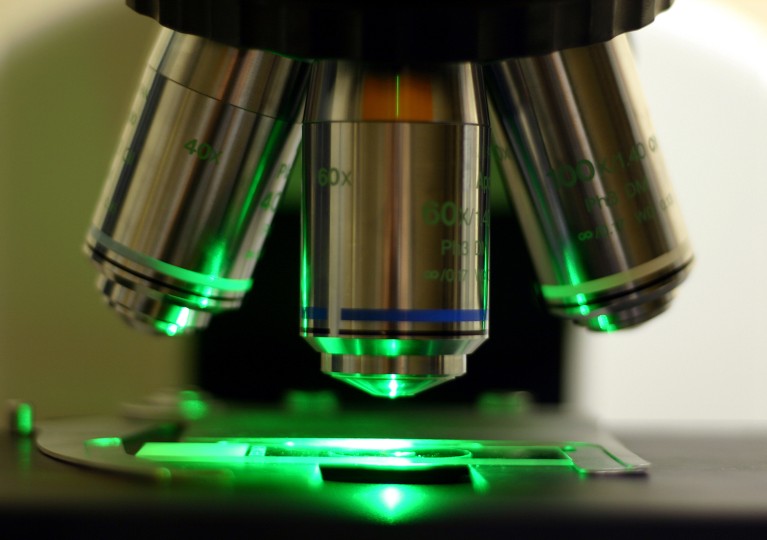
A molecular-tracking technique called PAPA-fSMT uses fluorescent tags to study protein complexes in the cell.Credit: Getty
Living cells teem with proteins. But those proteins rarely work alone; they drive cellular behaviour by pairing up with other proteins to form transient or long-lasting complexes. “Most proteins in the cell are not monogamous, so to speak,” says Thomas Graham, a biophysicist at the University of California, Berkeley (UC Berkeley).
Those relationships impact function: a protein can carry out several cellular tasks, depending on which molecules it interacts with.
But studying protein complexes in cells has been challenging. Most techniques require cells to be broken open to obtain proteins to study in a test tube, or molecules to be labelled — but often the labels are too sparsely distributed to observe interactions reliably. In 2022, Graham and his colleagues in the laboratory of biochemist Robert Tjian at UC Berkeley described a way to capture these interactions in live cells at the single-molecule level1. Called proximity-assisted photoactivation (PAPA), the method uses excitation of one fluorophore by green light to reactivate a nearby second fluorophore from a dark state. Most of the proteins that light up are in close proximity and so are likely to be in the same complex as the protein carrying the excited fluorophore. “PAPA, for the first time, lets us light up molecules that are interacting with a specific partner, and to look at those pairwise interactions within a cell,” says Graham.
New and improved
In a preprint posted on 25 June, the team builds on the technique by combining it with fast single-molecule tracking (fSMT) to pin down the molecular dynamics of a key step in the regulation of gene transcription2. They call the new method PAPA-fSMT.
The earlier work “was a very nice proof of concept, but here they moved it up a notch by adding single-molecule tracking so they could actually measure the diffusion of the protein complex as well as the individual protein”, says Sua Myong, a biophysicist at Harvard Medical School in Boston, Massachusetts, who was not involved in the study. “They show these kinetics in living cells for the first time.”
Graham says PAPA was discovered by accident during the COVID-19 lockdown. As part of a study of the nucleolus, a structure in the nucleus in which ribosomes are assembled, Graham was using certain wavelengths to flip between exciting a red fluorescent dye and a green one. As expected, the red molecules disappeared quickly owing to photobleaching, which changed their molecular structure such that they could not fluoresce. But as he continued alternating the wavelengths, the red dye reappeared — some of the particles had entered a dark state without being photobleached, and were apparently indirectly reactivated by the green-light excitation (see ‘Informative interactions’). Further studies revealed that, to be reactivated, a red-tagged protein had to be close to a green-tagged one, suggesting that the two proteins were likely to be bound up in the same complex. “That was very unexpected,” Graham says. “I’d never seen anything quite like it before.”
The reactivation signal was strongest when the molecules were in very close proximity — just 68 ångströms apart, or almost 3.5 times the diameter of the DNA double helix — but it remained detectable at more than 2.5 times this distance.
Although Graham has not yet characterized the full range of compatible dyes, he has seen the effect with rhodamine and carborhodamine dyes, as well as some fluorescent proteins. It’s not yet clear how exactly the reactivation process works — but the technique offers a valuable way to selectively investigate specific complexes in live cells, says Taekjip Ha, a biophysicist at Harvard Medical School.
Ha specializes in another approach for studying molecular proximity in cells: fluorescence resonance energy transfer (FRET), in which one fluorophore transfers its excitation energy to a nearby one. Energy transfer could explain why PAPA works, too, but the techniques operate over different distances: the FRET signal fades out at about half the distance of PAPA’s. Furthermore, in FRET, proteins are labelled so sparsely that the chance of catching two in complex with each other is much lower.
And, Graham notes, unlike with FRET, in PAPA the two dyes are excited at different times, so there’s no interference from background fluorescence. That’s important for single-molecule imaging at high protein concentrations.
Molecular dynamics
In the preprint, Graham and his colleagues explored how the kinase P-TEFb activates transcription. Normally, when RNA polymerase begins transcribing a gene, it pauses the process after transcribing the first few dozen bases; P-TEFb restarts transcription, but when it binds to the ribonucleoprotein complex 7SK, this activity is inhibited. The researchers labelled a subunit of P-TEFb, as well as HEXIM1, a subunit of the 7SK complex. The team applied PAPA to four pairs of proteins and found that the P-TEFb–7SK complex diffuses more slowly, consistent with its heavier molecular weight, but is most often not bound to chromatin — suggesting that generally the complex exists unbound to DNA. Adding a P-TEFb inhibitor to the system led P-TEFb and HEXIM1 to quickly dissociate. “We see that the whole complex synchronously falls apart when you treat the cells with this inhibitor,” Graham says.
Myong suggests several strategies for combining PAPA and single-molecule tracking in her own research on phase separation, a phenomenon in which intracellular molecules spontaneously separate into what are essentially distinct cellular compartments. The technique cannot reveal new players in the complexes she studies, because researchers must know the components’ identities to tag them, Myong notes. “But if you have the right proteins labelled, you could try to map those things one by one, which would be really cool.”
Meanwhile, Graham and his colleagues are continuing to build on PAPA to fill in the molecular details of transcription. Currently, Graham is using the technique to pin down not just whether molecules interact, but for how long they do so; he also aims to improve the technique’s specificity by developing ways to account for non-specific reactivation, which shows up as background noise that can muddy the picture.
New hardware could also help. Graham is collaborating with biophysicists Eric Betzig and Srigokul Upadhyayula and their postdoc Amir Hay at UC Berkeley to build a new type of microscope that can capture a larger number of trajectories of single molecules. “There’s this whole universe of protein interactions to explore in live cells,” Graham says. Now, thanks to PAPA-fSMT, researchers might finally be able to take a crack at studying them where they reside, instead of in test tubes.